Introduction
Microalgae are a valuable natural resource that have remarkable bioactive properties. Each species of microalgae produces different quantities of antioxidants (López-Hernández et al., 2020). It is well known that a broad spectrum of the compounds produced by microalgae possess antioxidant qualities. These substances include vitamins A, C, and E, polyphenols, carotenoids, and bioflavonoids, to name a few. Antioxidants are well known for their positive health effects and are essential for cells’ defense against free radicals (Sansone & Brunet, 2019). Euglena sp. is a single-cell alga that is edible and capable of producing carotenoids. There is an urgent need for technology that can identify and cultivate certain cells that produce more carotenoids since it facilitates the expensive and secure synthesis of these molecules (Yamashita et al., 2018).
The biomass of Euglena also contains relatively high concentrations of α-tocopherol, the most bioactive form of vitamin E (Ogbonna et al., 1998). The strongest known fat-soluble antioxidant in nature is alpha-tocopherol, one of the eight forms of vitamin E (Tucker & Townsend, 2005). Apart from facilitating immune responses in cells, α-tocopherol protects membranes from damage and regulates gene expression and signal transduction in both redox-dependent and redox-independent ways, managing cellular processes essential to its function and averting illnesses such as cancer, atherosclerosis, inflammation, and neurodegenerative disease (Zingg, 2019). Microalgae also include extra antioxidants that may be carotenoid. The primary function of the supplemental pigments known as carotenoids is to absorb light. Carotenoids can assist with photoprotection against UV damage and stabilize protein structures (Brunet et al., 2011). Carotenoids also play a critical role in mitigating the symptoms of a number of cancers, premature aging, cardiovascular diseases, and arthritis due to their anti-oxidant properties (Chen et al., 2017; Vershinin, 1999).
Current barriers to producing bioenergy from microalgae are expensive and low efficiency (Zhang et al., 2016). Using stressful circumstances, such as salt stress, can assist in overcoming it. Salt stress produces ionic and osmotic pressure, which presents unique challenges for species that rely on photosynthesis. Microalgae have evolved numerous physiological, metabolic, and molecular mechanisms to reduce these damages brought on by salt stress (Wang et al., 2018). Another effect of salt stress is the production of reactive oxygen species (ROS), which impede photosynthesis and threaten the growth and survival of organisms (Bazzani et al., 2021). The cell produces biomolecules such as tocopherol, polyphenols, carotenoids, flavonoids, and other enzyme scavengers including catalase, superoxide dismutase, and ascorbate peroxidase as an essential part of its internal defense system to regulate and reduce stress (Cirulis et al., 2013).
A significant financial and energy barrier to downstream microalgal processing is biomass dewatering and drying (Nazloo et al., 2022). Besides raising lipid biomass, salt stress has other benefits such as flocculation or harvesting. The effects of the added salts on algal excretion (dissolved organic content) may have a negative impact on the operation of separation of algal cells via membranes or settling (Discart et al., 2014). The current study aims to investigate the effects of different salts on growth, antioxidant biomolecules, and flocculation efficiency from Euglena sp., since there hasn’t been much research done yet on the effects of specific salinity stress on tocopherol content and flocculation efficiency in this species. The Euglena sp. used in this study was isolated in Central Java on the Dieng Plateau of Indonesia. There are various possible habitats for Euglena sp. on the Dieng Plateau, including peatlands and Telaga Warna Lake. Euglena sp. found in peatlands and many lakes on the Dieng plateau are the subject of few research at the moment. Consequently, studies on the isolation of Euglena sp. from the Dieng plateau and its analysis as antioxidant biomolecules were conducted.
Materials and Methods
This study was carried out at Universitas Gadjah Mada’s Biotechnology Laboratory in Yogyakarta. The microalgae employed is Euglena sp., and it was cultivated in Cramer-Mayers medium (CM) (Cramer & Myers, 1952) for 13 days after being collected from the Dieng Plateau in Central Java. 1 L culture container containing 600 mL of CM and 400 mL of inoculant Euglena sp. was already filled with the culture of Euglena sp. Each treatment was made in 3 replicates. The sterile bottles were incubated at 22 ± 2°C, 2,100 Lux of constant white fluorescent lighting, and pH 3.5. The salinity factor was used to condition the medium at three distinct salinities: 0 (control), NaCl 200 mM, and KCl 200 mM (Fig. 1).
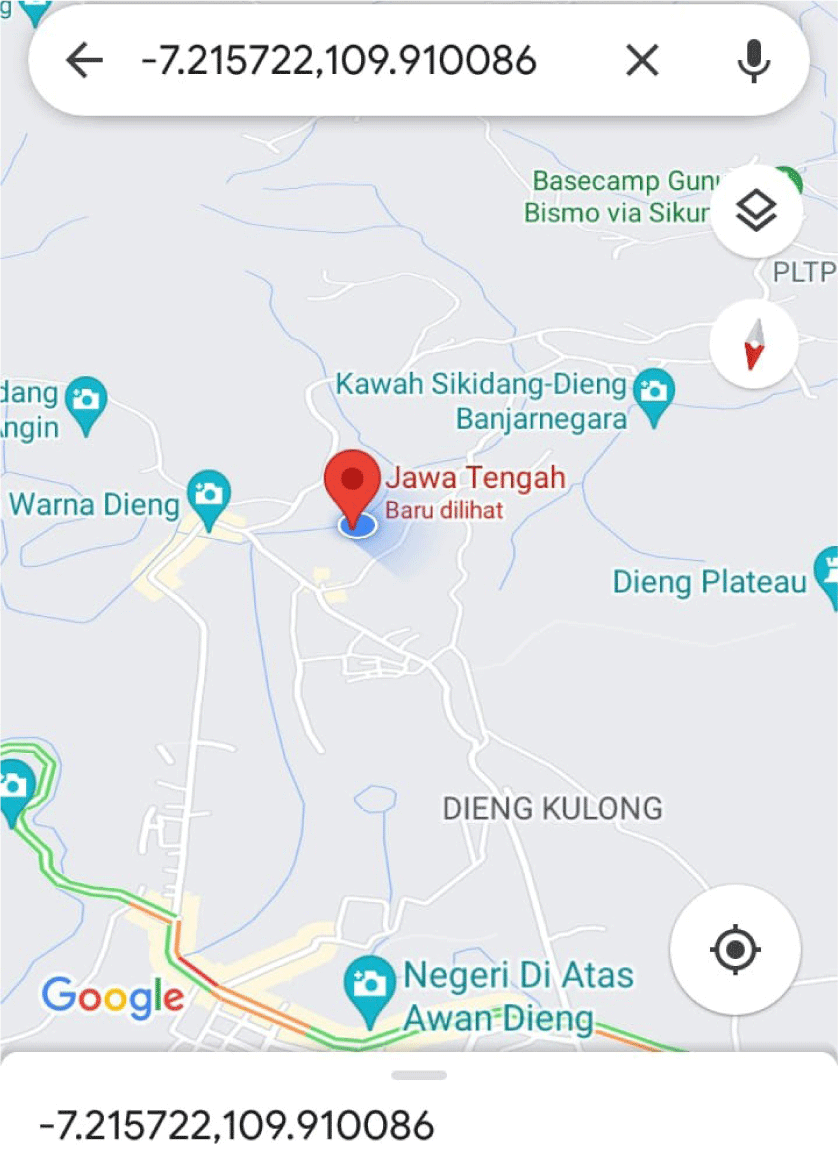
A light microscope (Olympus CX22LED, Tokyo, Japan) and Opti lab (Optilab Advance Lite, PT Miconos, Yogyakarta, Indonesia) were used to measure the amount of microalgal cells using a Neubauer haemocytometer (0.100 mm, Marienfeld Superior, Lauda-Königshofen, Germany). Alcohol 70% (Jakarta, Indonesia) was included in all treatments as a diluent. The data was calculated for the specific growth rates and the doubling time. Equation (1) was used to determine the specific growth rate:
where N1 and N2 are the initial and final cell density at initial time (T1) and final time (T2), respectively.
where μmax = Specific growth rate (d−1) and Dt = doubling time (d).
The 10 mL sample was transferred into a Buchner Funnel vacuum that had been lined with Glass Micro Fiber GF/C Whatman Filter Paper 1822-047 (Whatman, Buckinghamshire, UK) and rinsed with distillate water in order to extract the biomass. The harvested biomass was weighed after drying for 24 hours at 40°C. The following equation was used to calculate biomass productivity:
The growth patterns of Euglena sp. were modeled using the logistic and gompertz models. The logistics model was initially created using the following formula (Equations (4) and (5)), according to Phukoetphim et al. (2017) and Hanief et al. (2020), where X is cell density, Xo is initial cell density, Xmax is maximum cell density, and μmax is maximum specific growth rate.
The variables of the Gompertz model were maximum cell production (rm) and lag time (tL). Sum square total stands for SST, while sum square residual stands for SSR (Hanief et al., 2020; Phukoetphim et al., 2017). To establish the model, these formulas were employed.
α-Tocopherol was measured follow method from Afiukwa & Ogbonna (2007) with little modification. The sample was centrifuged for ten minutes at 1,968×g. After removing the supernatant, it was washed with distilled water. To extract the cell content, 2 mL of acetone (Merck, Darmstadt, Germany) was added and let to stand for 10 minutes. One millilitre of distilled water and 2.5 millilitres of petroleum ether (Cilacap, Indonesia) were then added. The top layer was gently collected with a pipette to read the absorbance at 450 nm with a UV/VIS spectrophotometer (Genesys, 150) to estimate the content of fat-soluble vitamin E. From the following equation, the vitamin’s cellular concentration was determined:
A = absorbance, DF = dilution factor, Ext. Coeff = absorption coefficient (1,026 for vitamin E in mg−1cm−1mL), light path = thickness of cuvette (usually 1 cm) and wgt = dry weight of cell used (gmL−1).
The method used to test the pigment content refers to the research of Thrane et al. (2015) and carried out by centrifuge model Thermo Fisher Scientific.
Pheophytin was determined by centrifuging two millilitres of microalgae culture at 17,709×g for five minutes, discarding the supernatant, and then mixing the algal pellet with 96% ethanol (Merck) and allowing it to sit for 24 hours at 4°C in the dark. Utilizing UV-vis spectrophotometry (Genesys 150) at 417 for pheophytin a and 437 nm for pheophytin b. After incubation overnight, the sample was assessed.
A total of 12 different forms of carotenoids were evaluated in this study, including 9-cis-neoxanthin, β, β-carotene, all-trans zeaxanthin, -cryptoxanthin, peridinin, antheraxanthin, trans-diadinoxanthin, dinoxanthin, diatoxanthin, fucoxanthin, and cis-dinoxanthin (Table 1). A maximum of 2 mL of each sample was obtained, and it was centrifuged at 17,709×g for 5 minutes. Pellets were then immersed in 96% ethanol and acetone from Merck (Germany) for up to 2 mL each, extracted from the supernatant, and incubated for 24 hours at 4°C in a refrigerator. After that, the resultant carotenoid derivatives were examined using the MetaboAnalyst 6.0 Program.
The concentration of each type of carotenoid is then calculated using the Lambert-Beer Law formula using the absorbance value obtained from the measurement of chlorophyll (Chl) and carotenoid derivatives (Lichtenthaler & Buschmann, 2001):
where Cw is the weight concentration (gL−1); Α is the absorbance; d is the cuvette groove length (cm) usually 1 cm; α is the specific absorbance coefficient (L.g−1.cm−1).
2 mL of the algal sample was transferring in a cuvette and measuring the cell density (OD680) after 0, 8, 24, and 48 hours, it was possible to determine the flocculation efficiency. Then, 10 mL of sample was placed into a 15 mL centrifuge tube (Onemed, Surabaya, Indonesia), and the precipitation rate was monitored every 0, 8, and 48 hours in order to visually see the deposition process. The efficiency of flocculation (Ef) was estimated using the equation where ODb is the cell density (optical density) prior to flocculation and ODa is the cell density following flocculation.
Microalgal cell shapes were measured with method from Jeon et al. (2019). Opti lab Viewer 4 and the BDS400 Inverted Biological Microscope (Chongqing Optec Instrument, Chongqing, China) were used to measure the dimension of microalgal cells at a 20-fold magnification. The average cell size per sample was 100 using image processing software (Image Raster 3).
Results and Discussion
Many different kinds of organisms, including microalgae, are subjected to severe stressors when exposed to salt. According to Nurafifah et al. (2023a), the microalga Euglena sp. have the capacity to serve as a sustainable and environmentally benign source of energy. Algal growth is seen to be declining with increasing salt concentrations, such as NaCl 200 mM and KCl 200 mM (Fig. 2A). High salinity causes hyperosmotic stress and ionic imbalance in photosynthetic organisms, according to Yokoi et al. (2002). When there is an ionic imbalance in microalgae, which is caused by an excess of Na+, K+, and Cl− and a decrease in the uptake of other mineral nutrients, ROS are formed (Das & Roychoudhury, 2014). Under high NaCl and KCl stress, the green alga Micrasterias denticulate even undergoes programmed cell death (PCD) (Affenzeller et al., 2009). Other research have shown that the highest salinity considerably reduced and hampered the growth of Phormidium versicolor, Dunaliella salina, and Cylindrotheca closterium (Guermazi et al., 2023).
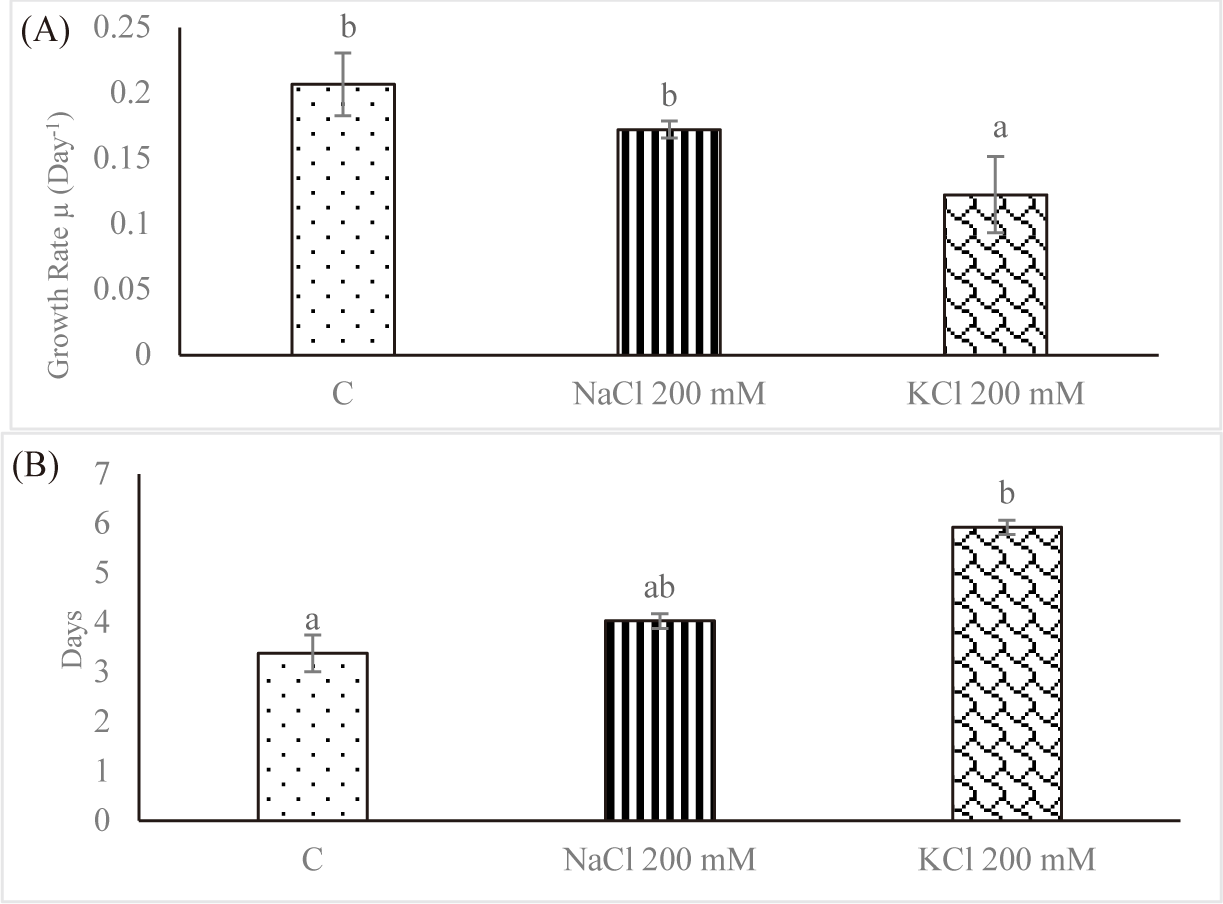
Since it reflects the growth dynamics of the microorganisms, the specific growth rate is the most crucial factor to be controlled throughout cultivation in vitro. The effect of salt stress on the average SGR of Euglena sp. is shown in Fig. 2A. For control, the average SGR was 0.206 ± 0.023 per day; for NaCl 200 mM and KCl 200 mM, it was 0.171 ± 0.006 per day and 0.122 ± 0.029 per day, respectively. As the salt concentration increased, the average SGR decreased. In contrast, Euglena sp. in the control group had a doubling time of 3.384 ± 0.372 days; however, doubling times rose to 4.033 ± 0.148 days and 5.927 ± 0.140 days, respectively, when the salinity was increased to NaCl 200 mM and KCl 200 mM (Fig. 2B). Church et al. (2017) found that salinity stress decreased the rate of algal growth in wastewater by 0.003 day−1 per mS/cm. In fresh wastewater, Chlorella vulgaris multiplied twofold in 2.5 days; however, growth required 13.6 days when the salinity was increased to 74 mS/cm.
Fig. 3 shows that there was no significant difference (p < 0.05) in the biomass production of Euglena sp. after being subjected to a salinity stress compared to cells cultivated in control, NaCl 200 mM, and KCl 200 mM. The next step is to use proper kinetic models to understand about the dynamics of microalgae biomass growth. Under the assumption of sufficient substrate, the Logistic model shows the evolution of numerous species as a function of growth rate, beginning biomass, maximum biomass concentration, and time, without accounting for substrate inhibition (Mu et al., 2006). Another well-used model for predicting microbial development as a function of time, productivity, and maximal biomass concentration is the modified Gompertz model (Eifert, 1994).
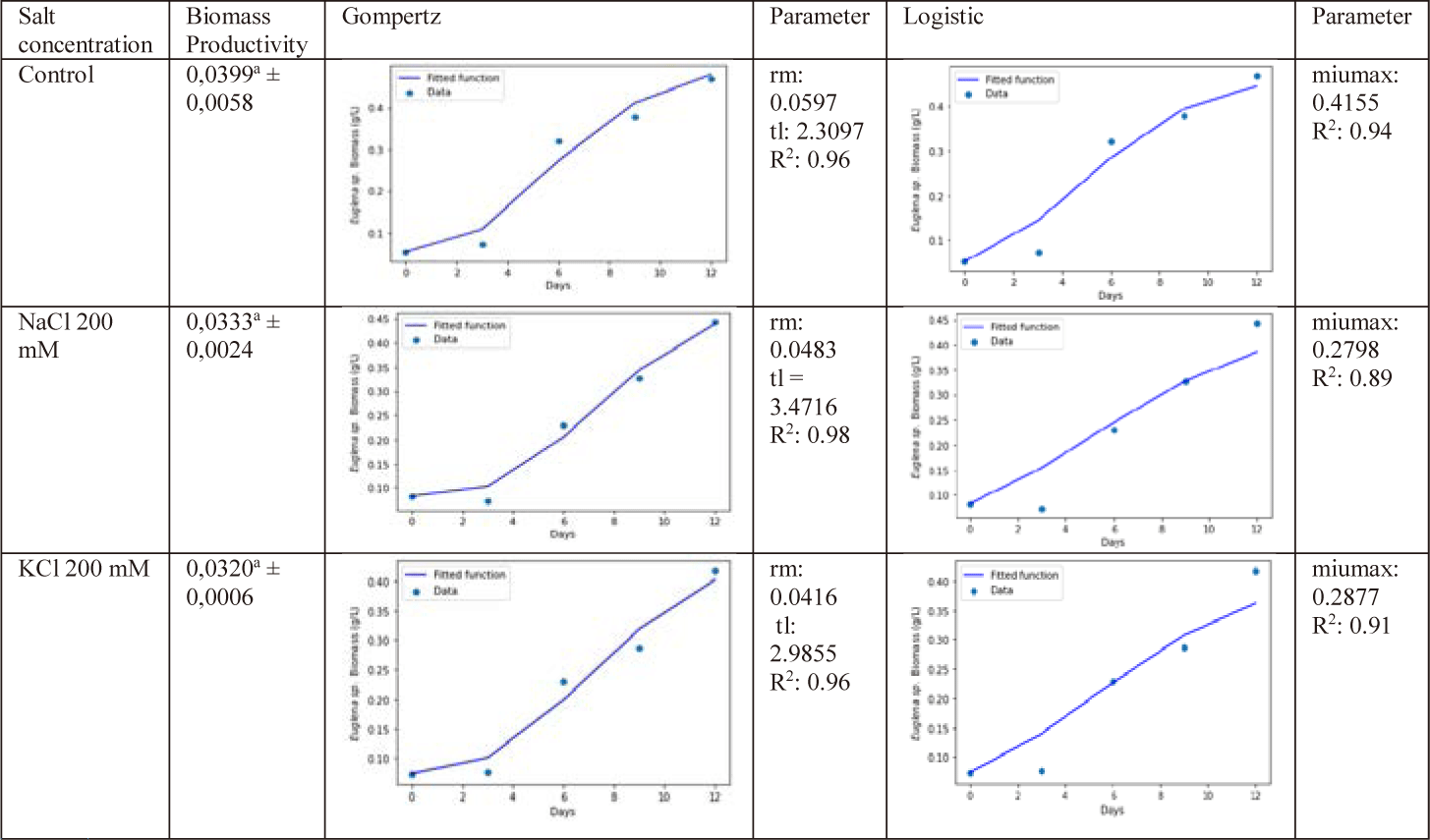
In this study, Gompertz and logistic models are employed, as Fig. 3 illustrates. The control group had the highest cell production rate (rm), 0.059, and the longest lag time (tL), 3.471. R squares were 0.96, 0.98, and 0.96 for the control, NaCl 200 mM, and KCl 200 mM, respectively. The maximum specific growth rates (max) for the control, NaCl 200 mM, and KCL 200 mM were, in accordance with logistic modeling, 0.415, 0.279, and 0.287/day, respectively. Then, the R2 error values were 0.94, 0.89, and 0.91. The table demonstrates that the Gompertz model more closely resembles the microalgae biomass curves than the Logistic model when microalgae are simulated under salt stress. In this study, the Gompertz model’s fit to the data was evaluated using the coefficient of determination (R2) values in comparison to the logistic model.
Fig. 4 displays the amount of α-tocopherol on Euglena sp. after increasing the salt stress. In comparison to the control group, the level of α-Tocopherol increased when subjected to salt stress (NaCl 200 mM and KCl 200 mM) with value of 90.393 ± 16.315 mg/g dry cell for control, 201.087 ± 16.513 mg/g dry cell for NaCl 200 mM and 137.910 ± 13.812 mg/g dry cell for KCl 200 mM. The treatment with NaCl 200 mM produced the highest concentration of α-tocopherol. That may occur when stress is high as a defensive mechanism.
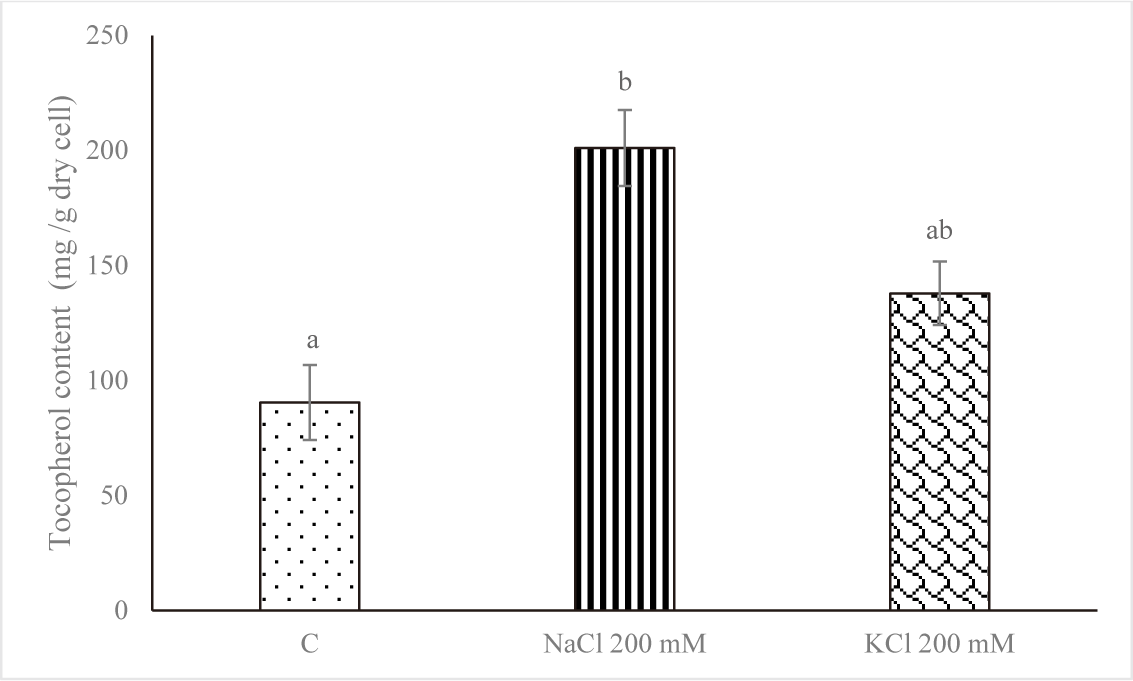
Of all the microorganisms, Euglena cells contain the highest proportion of alpha-tocopherol. Tocopherols are a class of antioxidants that can protect the body against many diseases caused by oxidative damage. The alpha-tocopherol isomer of tocopherol has the highest biological activity (Aqilla et al., 2023). The changes in degradation, recycling, and pathway gene expression are responsible for the substantial increase in tocopherol levels in response to environmental cues. Moreover, it was found that an increase in tocopherol accumulation contributes to stress tolerance by decreasing the generation of reactive oxygen species. Conversely, oxidative damage could arise from a decrease in tocopherol concentrations (Hasanuzzaman et al., 2014). Singh et al. (2022) report that Monoraphidium sp. CABeR41 accumulates lipids and total tocopherols due to NDHC and NLHC. Another study by Singh et al. (2020) reports that in nitrogen-depleted conditions, the total tocopherol content increased, rising from 638.2 to 1,450.24 µg/g dcw.
Fig. 5 demonstrates that the control group’s pheophytin concentration was the highest. NaCl 200 mM and KCl 200 mM treatments of salt stress reduced the amount of pheophytin. A significant variation was noted (p < 0.05) between the pheophytin level of the control and the concentrations of NaCl 200 mM and KCl 200 mM. Salt stress negatively affected cell growth and was linked to a drop in chl concentration, according to Kanna et al. (2021).
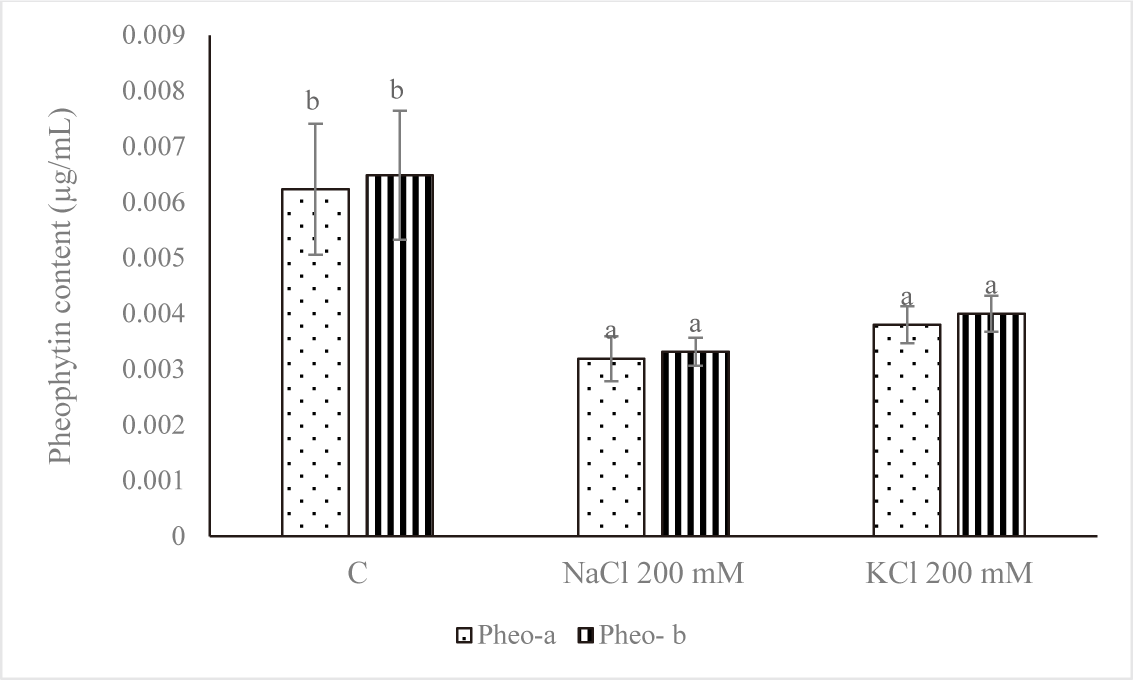
By releasing the phytol tail, the magnesium-deficient Chl derivative pheophytin can be further converted into pheophorbide (Indrawati et al., 2021). Pheophytins a (phe-a) and b (phe-b), which are the two primary derivatives of Chl, are two olive brown pigments produced when Mg2+ is removed from the porphyrin ring in an acidic environment (Kusmita et al., 2015). As an intermediary for the first electron carrier in the transfer route, pheophytins a and b in photosystem II plants are most likely structurally similar to the two Chls. As an intermediary for the first electron carrier in the transfer route, pheophytins a and b in photosystem II plants are most likely structurally similar to the two Chls (Eijckelhoff & Dekker, 1997).
The heatmap may display how the salt (NaCl and KCl) affects the pigment content of Euglena sp. Data shown in the heatmap indicate that some of the substances that are abundant in Euglena sp. are antheraxanthin, dinoxanthin, diatoxanthin, fucoxanthin, β-cryptoxanthin, all trans zeaxanthin, β-β caroten, peridinin, myxoxanthophyll, trans diadinoxanthin, and cis-Diadinoxanthin. This is in line with research from Nurafifah et al. (2023b), Euglena sp. contains the Chl pigments a and b along with carotenoids such as xanthophyll, astaxanthin, zeaxanthin, beta-carotene, and carotene. From this study the treatments with the highest pigment levels after the control treatment were KCl 200 mM and NaCl 200 mM. As the concentration of salt (NaCl 200 mM and KCl 200 mM, respectively) rises, the pigment content frequently decreases (Fig. 6).
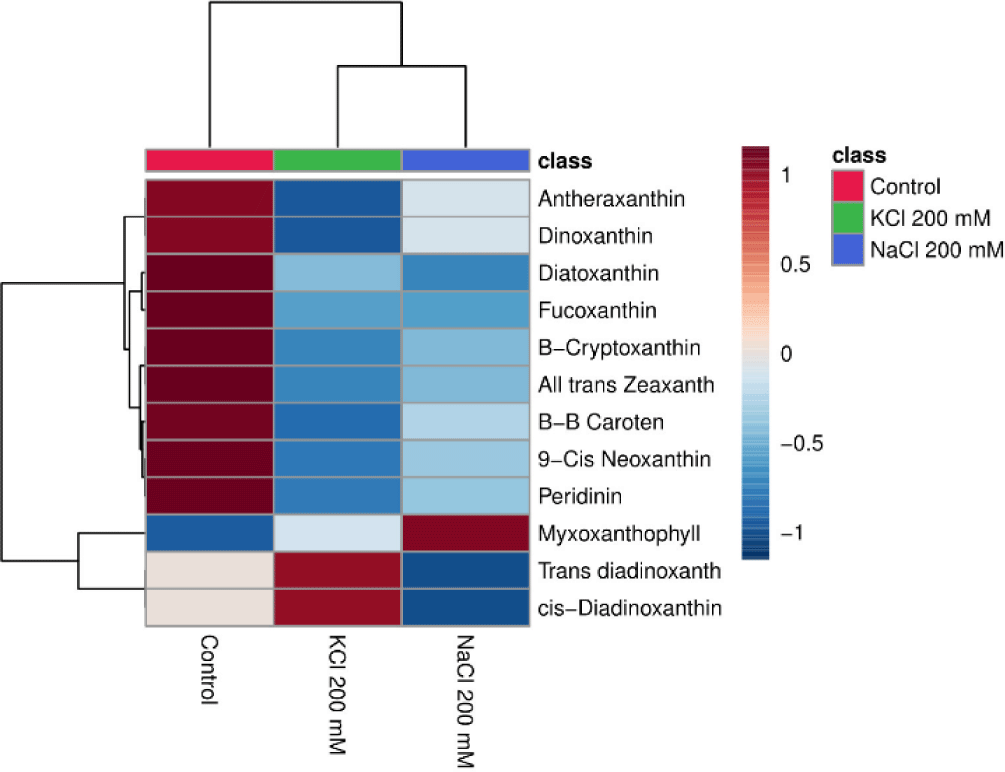
Principal component analysis (PCA) is an approach that’s widely used in unsupervised multivariate analysis to reduce dimensions (Liu et al., 2020). It makes it easier to discern the underlying patterns in the data and draws attention to the similarities and differences between samples of Euglena sp. treated with salinity. PC1 and PC2, at 59% and 17.9%, respectively, represent the variance. The loadings and scores for the two components are shown in Fig. 7. Three groups of metabolites were identified by PCA in the three different salt treatments (Control, NaCl 200 mM, and KCl 200 mM), indicating a significant difference in the secondary carotenoid content of Euglena sp.
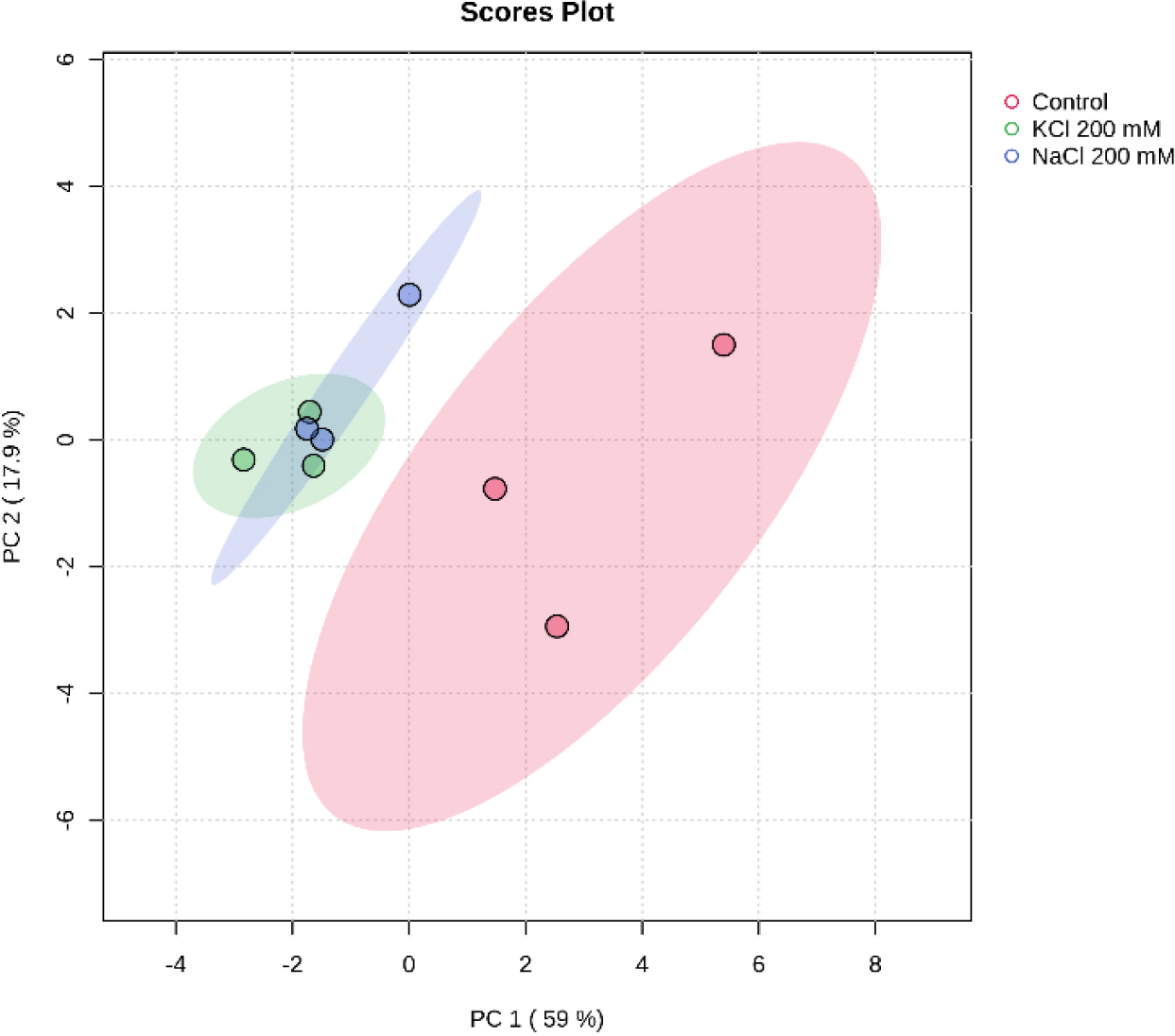
Harvesting and dewatering biomass are the most expensive steps in the production pipeline, excluding the cost of growing. This study demonstrated that salinity improved flocculation efficiency. The average percent flocculation efficiency rose every eight, twenty-four, and forty-eight hours for Euglena sp. grown in NaCl 200 mM and KCl 200 mM (Fig. 8). As seen on the tube, depositions took place over the first eight hours, and the amount of testimony increases over time. The rate of deposition was slower in the control treatment, and there was only a slight increase in deposition up to 48 hours (Fig. 9).
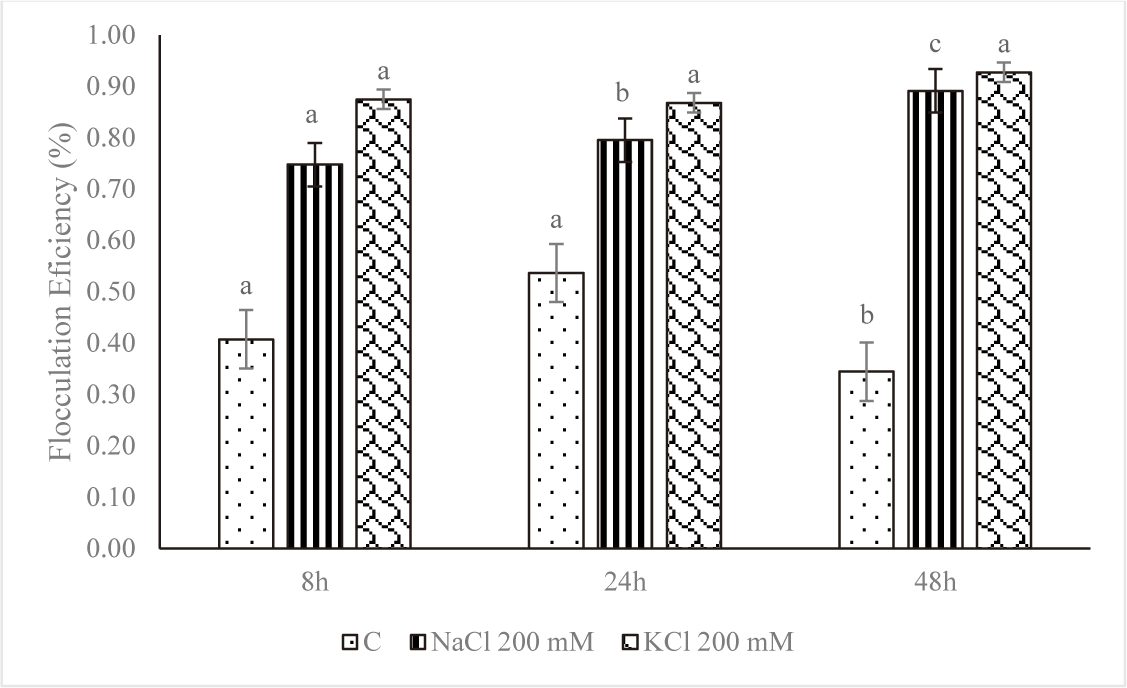
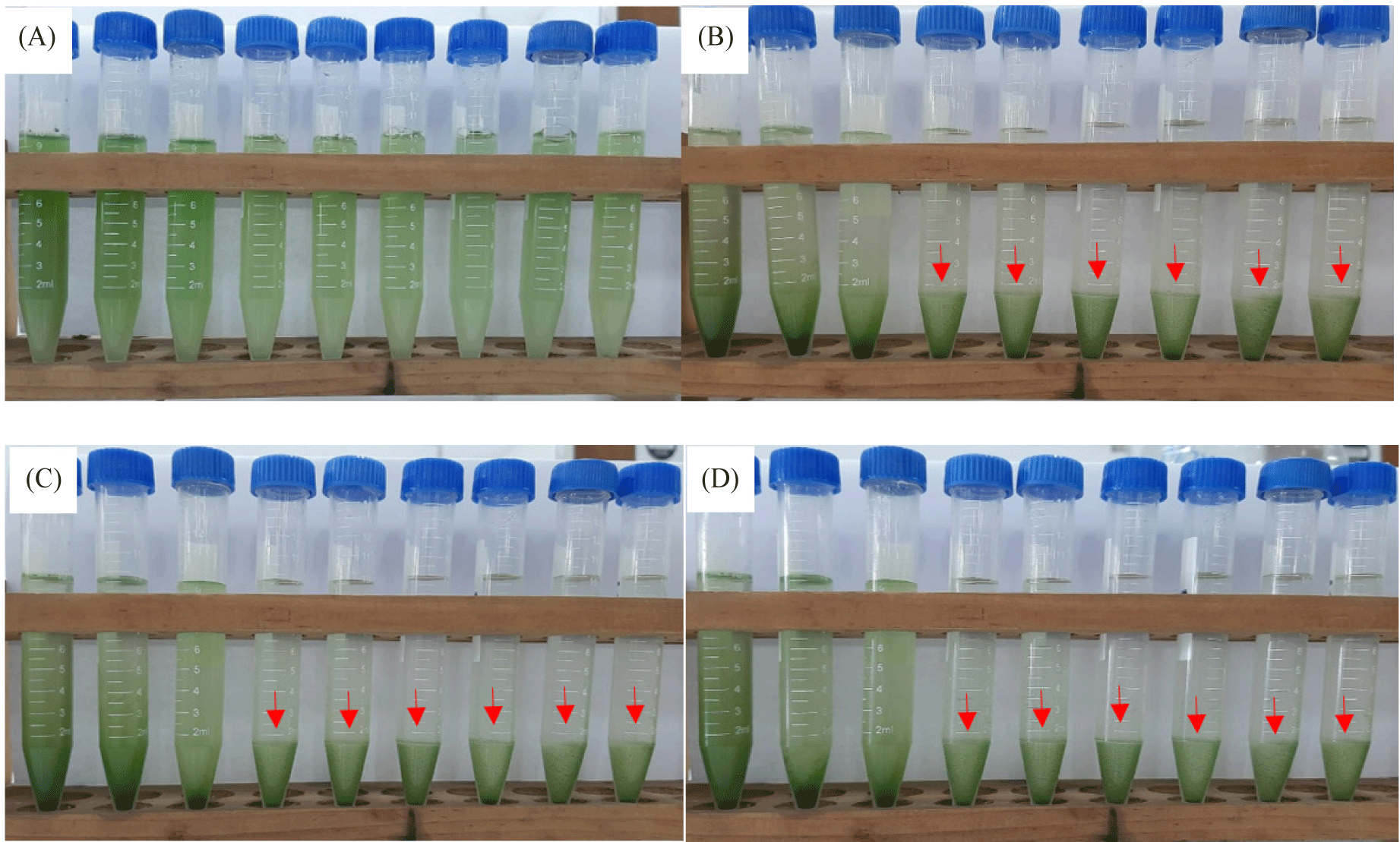
However, at higher salt concentrations, the ionic strength of saline liquids may affect the negative at the cell surface, causing microalgae to settle more quickly. The plasma membrane, cell wall, and other extracellular components would therefore have a lower zeta potential (Church et al., 2017; Hao et al., 2017). The addition of KCl and NaCl accelerated the settling rate, according to a study by Munshi et al. (2023). According to the study’s findings, microalgae settled more quickly after the addition of salts, NaCl 200 mM and KCl 200 mM, for the first eight hours of the growth of Euglena sp., the values were 0.75% and 0.87%, respectively. The reason for this could be because salt has the ability to raise the density and surface tension of water (Winkler et al., 2012), which increases the water’s resistance to C. vulgaris.
Table 2 and Fig. 10 show the morphological form and appearance of Euglena sp. after treatment with salt. The morphologies of Euglena species are ascertained using the aspect ratios (AR) given below: AR > 5, elongated; 1.5 < AR < 5, spindle-shaped; AR < 1.5, spherical. Li et al. (2017) state that Euglena sp. can be identified by its unique cell shape, which is indicative of its unique cell condition or physiology. To ascertain whether introducing salt stress could result in morphological alterations in Euglena sp. The diameters of Euglena sp. cells were measured under a microscope to ascertain the extent of distinct cellular morphologies over the duration of diverse cellular morphologies subjected to salinity treatment. It is interesting to note that the salt stress treatment significantly altered the size and shape of the Euglena sp. cells. When exposed to salt stress, more Euglena sp. cells grew more spherical than under control conditions. This outcome is in line with research by Farkas et al. (2023), which found that rising salt concentrations cause distinctive morphological structure alterations. When compared to the cells in the salt-free TAP medium, Chlorella cells showed a considerable increase in size (cell perimeter values increased by 150%) based on CLSM photos.
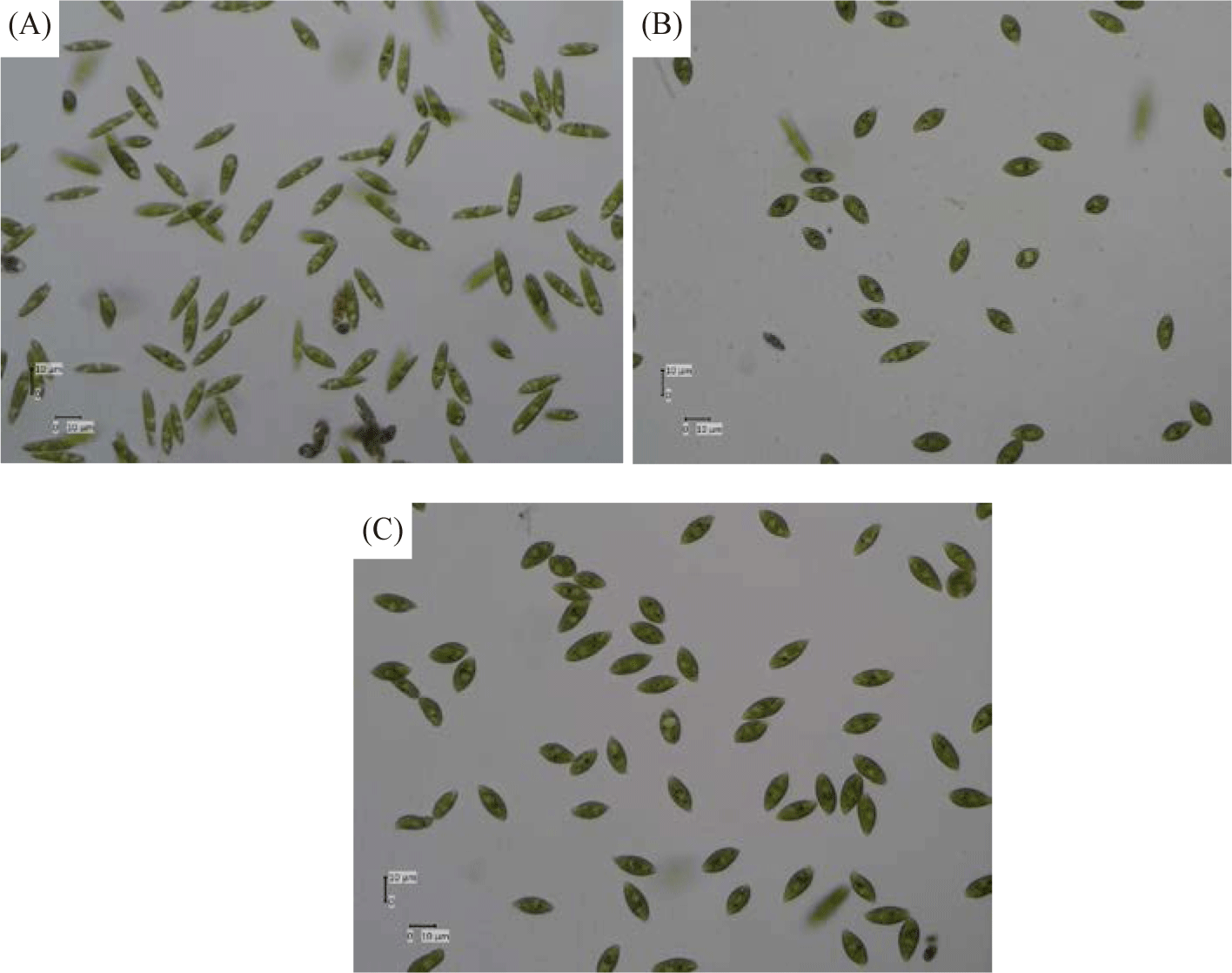
Cultured Euglena sp. in media containing NaCl 200 mM and KCl 200 mM show higher overall values of α-tocopherol content and flocculation efficiency when compared to other treatments. Twelve distinct types of carotenoids were found in this investigation. Antheraxanthin, dinoxanthin, diatoxanthin, fucoxanthin, β-cryptoxanthin, all trans zeaxanthin, β-β caroten, peridinin, myxoxanthophyll, trans diadinoxanthin, and cis-diadinoxanthin are among the several types of carotenoids. The use of natural antioxidants like Euglena sp. rather than synthetic antioxidants could be advantageous to the functional food and pharmaceutical industry. Further research is still needed to optimize antioxidant production in the microalgae Euglena sp. or other microalgae which are rich in carotenoid pigments and other antioxidant compounds. Through this study, it is also necessary to clarify the significance of this culture parameter on flocculation efficiency, which may be a crucial component in reducing the harvesting cost in microalgal production.