Background
Zinc (Zn) is an essential trace metal in fish, providing a vital structural and catalytic function to more than 300 proteins integral to piscine growth, reproduction, development, and immune function (Bury et al. 2003). However, Zn is released into aquatic environments as a result of industrial activities and exposure to high concentrations of Zn that induces toxicity in fish (Hogstrand et al. 2002).
Waterborne Zn induces histological aberrations in fish, such as leukocyte infiltration, epithelial cell proliferation, necrosis, hypertrophy, and mucus secretion (Wood 2001). In addition, Zn exposure is particularly toxic to fish as it inhibits calcium uptake, which creates an internal imbalance and results in hypocalcemia and disturbance of acid-base regulation (Santore et al. 2002).
Exposure to metal stimulates the production of reactive oxygen species (ROS), leading to oxidative metabolism injury and oxidative stress (Lushchak 2011). Exposure to Zn specifically leads to ROS generation, oxidant injury, excitation of inflammation, and cell death (Xia et al. 2008). The influence of metal exposure on ROS generation differs according to the exposure period and route of uptake (Coteur et al. 2005). ROS produced by aerobic organisms readily reacts with most biological molecules; thus, high concentrations of ROS production induce pathophysiological damage, such as arthritis, diabetes, inflammation, cancer, and genotoxicity (Senevirathne et al. 2006).
Non-specific immune responses in fish are considered a response to stressors, and metal exposure is associated with changes in the fish immune system (Kim and Kang 2016a). The non-specific immune system is considered a first line of defense against toxicants (Saurabh and Sahoo 2008). Given that immune parameters are influenced by aquatic toxins, metals, pesticides, hydrocarbons, and other chemicals, they can be used as important indicators to evaluate toxic effects in fish exposed to toxic substances.
Black sea bream, Acanthopagrus schlegelii, is a member of the family Sparidae. This species inhibits the inshore shelf of East Asia countries, such as South Korea and Japan at depths of 50 m. The species is omnivorous and protandrous, and breeds from February to May, dopositing eggs in the demersal zone. A. schlegelii is one of the most commonly cultured fish species and highly sought after in South Korea. The optimum temperature for growth and development of A. schlegelii is 18 °C; however, during the Korean summer, seawater temperature reaches 26 °C. The degree of waterborne Zn-induced toxicity in aquatic animals depends on water temperature and water chemistry, as well as the life stages of individuals (McGeer et al. 2000). However, information on the relationship between Zn toxicity and water temperature is scarce. Therefore, the present study evaluated the toxic effects of exposure to waterborne Zn and the influence of water temperature on Zn-induced toxicity using the antioxidant and non-specific immune responses of A. schlegelii.
Methods
Black sea breams, Acanthopagrus schlegelii, were obtained from a local fish farm in Tongyeong, Korea. Fish were acclimatized for 3 weeks under laboratory conditions (Table 1). Fish were fed a commercial diet twice daily (Woosungfeed, Daejeon City, Korea). After acclimatization, 72 fish (body length, 17.8 ± 1.5 cm; body weight, 96.7 ± 6.8 g) were selected for study. Waterborne Zn exposure took place in 20-L glass tanks, containing 6 fish per treatment group. Water temperature was adjusted from ambient at a rate of ±1 °C/day until a final temperature of 26 °C was reached. The acclimation period commenced once the final temperature had been sustained for 24 h. Zinc oxide (Sigma, St.Louis, MO, USA) solution was dissolved in respective glass tanks. Zn concentrations were 0, 200, and 400 μg/L (at 18 and 26 °C). An extremely high concentration of 400 μg/L Zn is much higher than that in nature, but this exposure experiment focused on Zn toxicity in experimental fish. Glass tank water was completely replaced once per 2 days and made the same concentration in respective glass tank. At the end of each period (at 2 and 4 weeks), fish were anesthetized in buffered 3-aminobenzoic acid ethyl ester methanesulfonate (Sigma Chemical, St. Louis, MO).
Exposure seawater (μg/L) | ||||||
---|---|---|---|---|---|---|
Concentrations (μg/L) | 0 (18 °C) | 0 (26 °C) | 200 (26 °C) | 200 (26 °C) | 400 (18 °C) | 400 (26 °C) |
Zinc concentrations | 0 | 0 | 200 | 200 | 400 | 400 |
Actual zinc levels | 8.6 | 9.8 | 212.5 | 208.7 | 421.3 | 418.5 |
Seawater samples were digested in 65% (v/v) HNO3 and dried at 120 °C. The procedure was repeated until total digestion. The entirely digested samples were diluted in 2% (v/v) HNO3. The samples were filtered through a 0.2-μm membrane filter (Advantec mfs, Ins.) under pressure for analysis. For determination of total Zn concentrations, the digested and extracted solutions were analyzed by ICP-MS. The ICP-MS measurements were performed using an ELAN 6600DRC ICP-MS instrument with argon gas (Perkin-Elmer). Total Zn concentrations were determined by external calibration. ICP multi-element standard solution VI (Merck) was used for standard curve. The Zn concentrations were expressed as microgram per liter (Table 1).
Liver and gill tissues were excised and homogenized with 10 volumes of ice-cold homogenization buffer using Teflon-glass homogenizer (099CK4424, Glass-Col, Germany). The homogenate was centrifuged at 10,000g for 30 min under refrigeration, and the obtained supernatants were stored at −80 °C for analysis.
Superoxide dismutase (SOD) activity was measured with 50% inhibitor rate about the reduction reaction of WST-1 using SOD Assay kit (Dojindo Molecular Technologies, Inc.). One unit of SOD is defined as the amount of the enzyme in 20 μl of sample solution that inhibits the reduction reaction of WST-1 with superoxide anion by 50%. SOD activity was expressed as unit mg protein−1.
* WST-1 = 2-(4-lodophenyl)-3-(4-nitrophenyl)-5-(2,4-disulfophenyl)- 2H -tetrazolium, monosodium salt Glutathione-S-transferase(GST) activity was measured according to the method of modified Habig et al. (1974). The reaction mixture consisted of 0.2 M phosphate buffer (pH 6.5), 10 mM glutathione (GSH) (Sigma), and 10 mM 1-chloro-2,-dinitrobenzene, CDNB (Sigma). The change in absorbance at 25 °C was recorded at 340 nm, and the enzyme activity was calculated as 340 nm, and the enzyme activity was calculated as nmol min−1 mg protein−1.
Reduced glutathione was measured following the method of Beutler (1984). Briefly, 0.2 ml fresh supernatant was added to 1.8 ml distilled water. Three milliliters of the precipitating solution (1.67 g metaphosphoric acid, 0.2 g EDTA, and 30 g NaCl in 100 ml distilled water) was mixed with supernatants. The mixture was centrifuged at 4500g for 10 min. 1.0 mL of supernatant was added to 4.0 ml of 0.3 M NaHPO4 solution, and 0.5 mL DTNB (5,5′-dithiobis-2-nitrobenzoic acid) was then added to this solution. Reduced glutathione was measured as the difference in the absorbance values of samples in the presence and the absence of DTNB at 412 nm. GSH value was calculated as μmol mg protein−1 in the tissues.
Blood samples were collected within 35–40 s through the caudal vein of the fish in 1-ml disposable heparinized syringes. The blood samples were centrifuged to separate serum from blood samples at 3000g for 5 min at 4 °C. Kidney tissues were obtained using sterilized dissecting tools, and the excised tissues were homogenizing with 10 volumes of ice-cold homogenization buffer (0.004 M phosphate buffer, pH 6.6) using Teflon- glass homogenizer (099CK4424, Glass-Col, Germany). Homogenate was centrifuged at 10,000g for 10 min under refrigeration, and the obtained supernatant was stored at −70 °C for analysis. Protein content was determined by the Bio-Rad Protein Assay Kit (Bio-Rad Laboratories GmbH, Munich, Germany) based on Bradford dye-binding procedure, using bovine serum albumin as standard.
Phagocytosis was measured with phagocytosis assay kit (Cell biolabs, Inc.). Briefly, we added 10 μl of Escherichia coli suspension in 100 μl plasma sample of 96-well plate. The samples were mixed well, and we immediately transferred the plate to a cell culture incubator at 37 °C for 3–6 h. Each sample including a negative control without E. coli particles was assayed in duplicate. After, we added 200 μl of cold 1× PBS to each well and promptly removed PBS solution by centrifugation and gentle aspiration. We added 100 μl of fixation solution by centrifugation and gentle aspiration and then washed the sample twice with 1× PBS. We added 100 μl of prediluted 1× blocking solution to each well, incubated the sample for 30 min at room temperature on an orbital shaker, and promptly removed blocking solution by centrifugation and gentle aspiration. The sample was washed three times with 1× PBS. We added 100 μl of prediluted 1× permeabilization solution to each well, and incubated the sample 5 min at room temperature. We promptly removed permeabilization solution by centrifugation and gentle aspiration, and the sample was washed twice with 1× PBS. We promptly removed the PBS by centrifugation and gentle aspiration, initiated the reaction by adding 100 μl of substrate, and incubated the sample for 10–30 min at room temperature. We then stopped the reaction by adding 100 μl of the stop solution and mixed it by placing the plate on an orbital plate shaker for 30 s. Finally, we read the absorbance of each well at 450 nm.
Lysozyme activity was determined by a turbidimetric method (Ellis 1990) using Micrococcus lysodeikticus (Sigma) as substrate (0.2 mg/ml 0.05 M phosphate buffer, pH 6.6 for kidney sample and pH 7.4 for plasma). A standard curve was made with lyophilized hen egg white lysozyme (Sigma), and the rate of change in turbidity was measured at 0.5-min and 4.5-min intervals at 530 nm. The results were expressed as microgram per milliliter and microgram per gram equivalent of hen egg white lysozyme activity (Anderson and Siwicki 1994).
The experiment was conducted in exposure period for 4 weeks and performed triplicate. Statistical analyses were performed using the SPSS/PC+ statistical package (SPSS Inc, Chicago, IL, USA). Significant differences between groups were identified using one-way ANOVA and Tukey’s test for multiple comparisons or Student’s t test for two groups. The significance level was set at P < 0.05.
Results
Antioxidant responses such as SOD activity, GST activity, and GSH concentration were analyzed to assess the oxidative stress by the waterborne Zn exposure depending on water temperature. Liver and gill SOD activity of the A. schlegelii is presented in Fig. 1. The liver SOD activity was significantly increased in 400 μg/L Zn at 18 °C and in 100 and 200 μg/L Zn at 26 °C after 2 weeks. After 4 weeks, a significant SOD activity in the liver was observed in the fish exposed to waterborne Zn greater than 200 μg/L at 18 and 26 °C. The gill SOD activity was substantially increased in 400 μg/L Zn at 26 °C after 2 weeks, and there was no significant alteration at 18 °C after 2 weeks. After 4 weeks, the gill SOD activity was notably increased in 400 μg/L Zn at 18 and 26 °C.
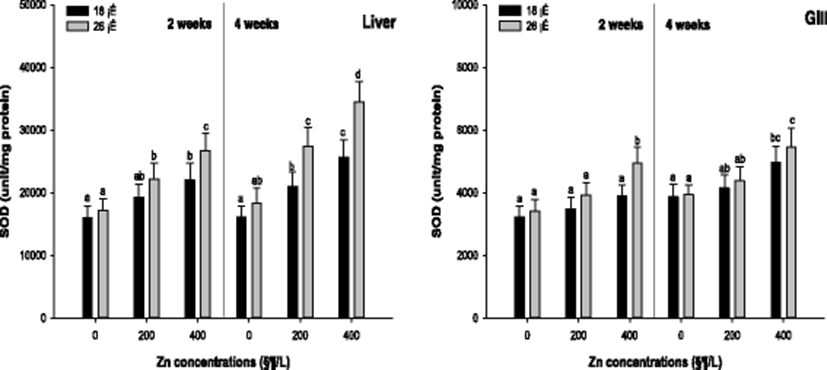
Liver and gill GST activity of the A. schlegelii is presented in Fig. 2. A significant decrease in the liver GST activity was observed in 400 μg/L at 18 °C and in 200, 400 μg/L at 26 °C after 2 weeks. After 4 weeks, the liver GST was significantly decreased in 400 μg/L at 18 and 26 °C. In the gill tissue, the GST activity was notably decreased in 400 μg/L at 18 and 26 °C after 2 and 4 weeks.
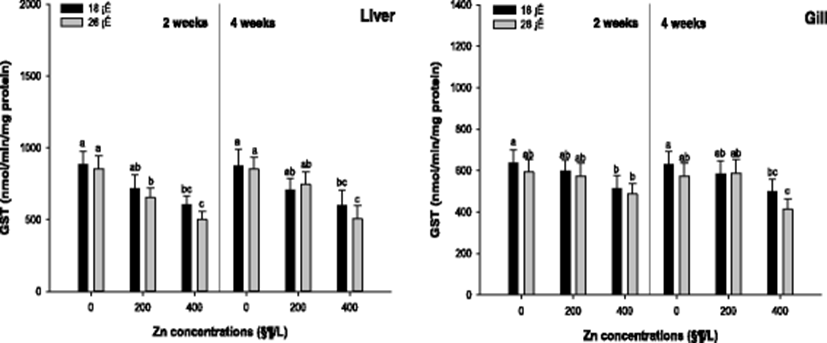
Liver and gill GSH activity of the A. schlegelii is demonstrated in Fig. 3. The liver GSH concentration was substantially decreased in 400 μg/L Zn at 18 and 26 °C after 2 weeks. After 4 weeks, a significant decrease in the liver GSH concentration was observed in the concentration of 400 μg/L Zn at 18 °C and 200, 400 μg/L Zn at 26 °C. The gill GSH concentration was notably decreased in 400 μg/L Zn at 18 and 26 °C. After 4 weeks, the gill GSH concentration was significantly decreased in 400 μg/L Zn at 18 °C and 200, 400 μg/L Zn at 26 °C.
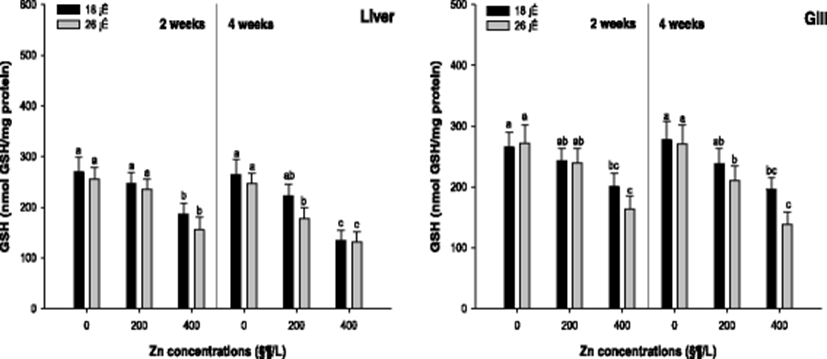
Non-specific immune responses such as phagocytosis and lysozyme activity were analyzed to evaluate the effects on the immune responses by the waterborne Zn exposure depending on water temperature. Plasma and kidney phagocytosis activity of the A. schlegelii is shown in Fig. 4. The phagocytosis activity in the plasma was significantly increased in 400 μg/L at 18 and 26 °C after 2 weeks. After 4 weeks, the phagocytosis activity was substantially increased in 400 μg/L at 18 and 200, and 400 μg/L at 26 °C. The phagocytosis activity in the kidney was significantly increased in 400 μg/L at 18 and 200, and 400 μg/L at 26 °C after 2 weeks. After 4 weeks, a notable increase in the phagocytosis activity was observed in 400 μg/L at 18 and 26 °C.
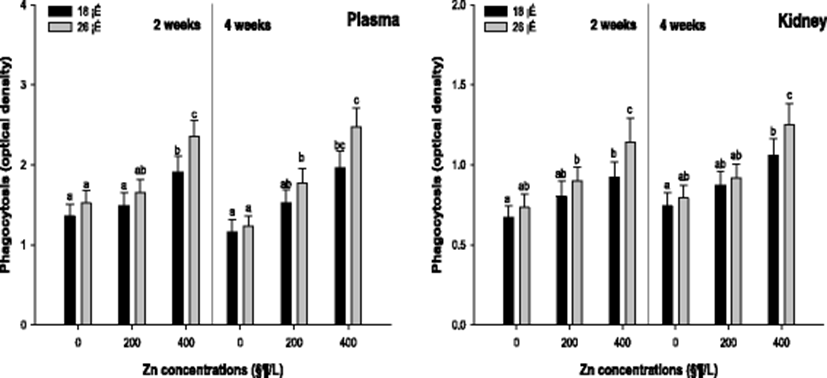
Plasma and kidney lysozyme of the A. schlegelii is demonstrated in Fig. 5. The lysozyme activity in the plasma was significantly increased in 400 μg/L at 26 °C after 2 weeks. But, there was no notable change at 18 °C after 2 weeks. After 4 weeks, a significant increase in the lysozyme activity was observed in 400 μg/L at 18 and 26 °C. The lysozyme activity in the kidney was also significantly increased in 400 μg/L at 18 and 26 °C after 2 and 4 weeks.
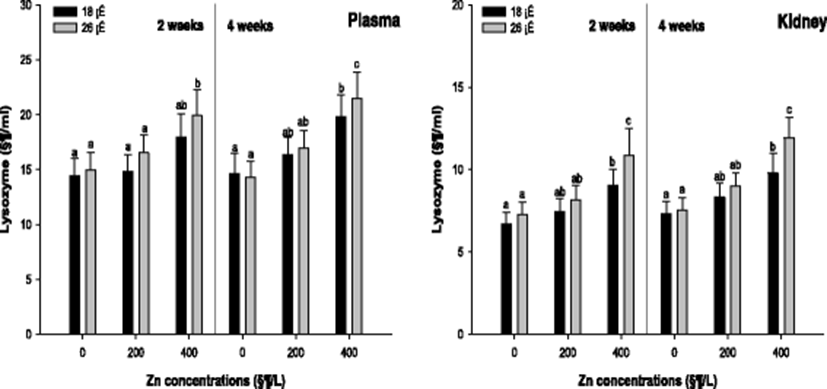
Discussion
Oxygen is an essential component of various metabolic processes in aerobic organisms. However, organisms that are reliant on oxygen must also resist its toxicity, as concentrations of ROS cause substantial damage to cell structures (Ahmad et al. 2004). Similarly, high concentrations of metal exposure cause redox reactions, free radical production, and ROS in fish tissues (Brucka-Jastrzebska 2010). Liver and gill tissues are generally used to assess antioxidant responses for oxidative stress (Kim and Kang 2016b; Kim et al. 2017; Kim and Kang 2017).
Several antioxidant responses are observed in fish, for example, superoxide dismutase (SOD), which catalyzes the transformation of superoxide anion to hydrogen peroxide (H2O2) (Ozturk-Urek et al. 2001). In the present study, exposure to waterborne Zn resulted in significant increases in SOD activities in the liver and gill of A. schlegelii. Farombi et al. (2007) also reported significant increases in the SOD activity in African cat fish, Clarias gariepinus, exposed to metals and a metalloid, including zinc, copper, cadmium, arsenic, and lead. Accumulation of metal may generate superoxide anions, which activates SOD to scavenge superoxide radicals. Glutathione-S transferase (GST) catalyzes the conjugation of glutathione (GSH) to various electrophiles and functions as a critical defense mechanism against ROS and xenobiotics (White et al. 2003). In this study, exposure to waterborne Zn significantly decreased GST activity in A. schlegelii. Significant decreases in the GST activity have also been reported in Nile tilapia, Orechromis niloticus, exposed to copper, with the removal of ROS by other enzymes in the antioxidant system possibly compensating for GST (Kanak et al. 2014). GSH, a thiol-containing peptide associated with cellular defense against the toxic effects of xenobiotics, such as metals, is a known substrate for GST activity (Lange et al. 2002). Pandey et al. (2008) reported a significant decrease in GSH levels in spotted snakehead, Channa punctate, that were exposed to multiple metals. Decreased GSH concentrations were related to decreases in GSH-dependent enzymes, such as GST, glutathione reductase (GR), and glutathione peroxidase (GPx). Sanchez et al. (2005) suggested that GSH concentrations are reduced by a cellular response that chelates and detoxifies metals, protecting cells from metal exposure. Loro et al. (2012) reported that exposing killifish, Fundulus heteroclitus, to waterborne Zn induced oxidative stress and changes in antioxidant enzymes; the release of Zn ions triggered the increased expression of genes coding for antioxidant enzymes. Similar to previous studies, exposure to waterborne Zn induced significant changes in antioxidant responses in A. schlegelii in this study. Based on these results, exposure to waterborne Zn should manifest in the experimental fish as oxidative stress.
Temperature is an important factor affecting oxidative stress, and a higher temperature can amplify oxidative stress (Lushchak and Bagnyukova 2006). Kim et al. (2007) reported that thermal stress, combined with metal exposure, induced significant increases in SOD activity in the disk abalone, Haliotis discus discus. Similarly, thermal stress significantly decreased GST in Channa punctata, as the elevated temperature decreased GSH concentrations (Kaur et al. 2005). Moreover, in O. niloticus, waterborne arsenic significantly decreased gill GSH, and this effect was more pronounced at a higher temperature (Min et al. 2014).
Several studies have indicated that metal exposure affects various parameters in the host immune system, increasing susceptibility to infection and allergy (Bernier et al. 1995). In addition, Arunkumar et al. (2000) suggested that metal exposure induces immune responses in fish, either directly, by binding the tertiary structures of biologically active molecules, or indirectly, by acting as stressors that influence corticosteroid concentrations. Kidney and plasma function in immune systems, and the samples are generally used to assess immune responses(Kim and Kang 2015; 2016c; 2016d; 2016e).
Phagocytosis is an important immune response wherein pathogenic particles are engulfed by intracellular vacuoles, and, removed. Therefore, it is a critical immunological parameter for evaluating the health status and immunity of fish exposed to toxicants (Risjani et al. 2014; Nagasawa et al. 2015). In this study, phagocytosis activity in A. schlegelii significantly increased with exposure to waterborne Zn. Pillet et al. (2000) also reported substantial increases in phagocytic activity in harbor seals, Phoca vitulina, and grey seals, Halichoerus grypus, exposed to Zn. Of the various non-specific immune responses, lysozyme is a key component of the innate immune response in fish and is stimulated by exposure to aquatic toxins (Bols et al. 2001). In this study, exposure to waterborne Zn caused a significant increase in lysozyme activity in A. schlegelii. Sanchez-Dardon et al. (1999) also reported notable elevations in the serum lysozyme in rainbow trout, Oncorhynchus mykiss, exposed to Zn, cadmium, mercury, and metal mixtures. Celik et al. 2012 reported increases in both phagocytic and lysozyme activity in Mozambique tilapia, Oreochromis mossambicus, exposed to Zn. Given that lysozyme is a reliable parameter for monitoring the influence of environmental changes in innate immunity in fish (Bols et al. 2001), our results suggest that exposure to waterborne Zn can be considered an environmental stressor for A. schlegelii.
In the present study, a combination of high temperature (26 °C) and Zn concentration significantly affected the immune responses of A. schlegelii. As fish are ectothermic, their physiology and immune function is directly affected by water temperature (Morvan et al. 1998). Parry and Pipe (2004) also reported a significant increase in phagocytosis in the blue mussel, Mytilus edulis, at a higher temperature. Furthermore, high water temperatures were associated with increased lysozyme activity in Pacific abalone, Haliotis discus hannai, exposed to nickel.
Conclusions
In conclusion, exposure to waterborne Zn significantly affected antioxidant responses (SOD, GST, and GSH) in A. schlegelii. Non-specific immune responses such as phagocytosis and lysozyme activity were also substantially influenced by exposure to waterborne Zn. Rising water temperatures from global warming may exacerbate the seasonal increases in water temperature in Korea, and changes in water temperature are an important aspect of waterborne Zn toxicity in these coastal waters. The results of the present study indicate that exposure to waterborne Zn affects fish such as A. schlegelii, and that water temperature is a critical factor in the toxicity of waterborne Zn.