Introduction
Yellowtail (Seriola quinqueradiata) is one of the most popular and important aquaculture species in Japan. The fish muscles are composed of dorsal ordinary muscle (OM) and dark muscle (DM), with different characteristics with respect to their texture and taste. Furthermore, as OM and DM are generally consumed simultaneously, the properties of each muscle type can influence the fish quality.
To obtain high-quality food products, it is necessary to control, factors such as texture, flavor, taste, and colour (Ishiwatari et al., 2013a). In addition, sufficient heating of food is required to minimize the risk of food poisoning. Therefore, suitable heating is required to maintain safety and palatability.
During heating of fish and meat, both textural changes and muscle fibre shrinkage occur due to the heat denaturation of muscle proteins, such as myosin, actin, and collagen (Ishiwatari et al., 2013a; Wattanachant et al., 2005). These changes affect the taste, juiciness, and texture of fish and are consequently related to fish product quality.
Freeze-induced denaturation of fish muscle proteins changes their chemical and physical properties (Sotelo et al., 1995). On the other hand, freezing also minimizes the deterioration of fish quality during storage (Konno, 2017). Frozen-thawed fish are generally used in the seafood processing industry and for daily cooking. Thus, the effect of heat treatment on the quality of frozen-thawed fish needs to be studied.
Several studies have reported changes in the texture of fish that vary with heating temperature and time (Kanoh et al., 1988; Qixing et al., 2014), and even sous-vide cooking (Fagan & Gormley, 2005; Llave et al., 2018). However, as there had been no reports on the effects of heating conditions designed to prevent food poisoning on the texture of fish, we analysed the texture of OM and DM in yellowtail heated under different heating conditions (Furuta et al., 2020). Our results suggested that heating conditions affect the hardness and sample thickness of the OM and DM and that, in particular, low-temperature/long-time pasteurization (LTLT) results in more tender fish with less shrinkage. However, the mechanism of these textural changes under different heating conditions is still unclear.
In order to clarify the mechanism of these textural changes under different heating conditions set to prevent food poisoning, we investigated the effect of heating conditions on both the solubility and protein composition in OM and DM of yellowtail. In addition, we used differential scanning calorimetry (DSC) to evaluate the thermal denaturation of fish proteins, and we determined the collagen solubility of OM and DM after heating.
Materials and Methods
Sample was prepared and heat treatments were performed according to a previous study (Furuta et al., 2020). Cultured yellowtail (Seriola quinqueradiata) (n = 3) were purchased with an average weight of 3.2 ± 0.5 kg. Fish were then filleted and skinned, and the fillet was sliced to a 20 mm thickness (94.4 ± 9.8 mm length × 34.3 ± 4.4 mm width). Sliced samples were wrapped in a polyethylene film, frozen in a blast chiller/freezeOsaka, Japan), and stored atr (iRiNOXAL-5M, FMI, Osaka, Japan), and stored at –80°C under atmospheric conditions until heat treatment. Before the heat treatment, the samples were thawed in an ice-water slurry for 1 h and stored at 4°C. The samples were heated in a steam convection oven (ACO-060GS, AIHO, Aichi, Japan) in steam mode at 100% humidity. Each part of the yellowtail was heated under the following heating conditions: (1) heating until the internal core temperature of the sample reaches 85°C followed by maintaining the temperature for 90 s (to inactivate norovirus for bivalve molluscs (FAO & WHO, 2012) ; 85°C, high-temperature/short-time heating [HTST]) (2) heating until the internal core temperature of the sample reaches 75°C followed by maintaining the temperature for 60 s (to kill foodborne pathogens, such as Escherichia coli O157 for meat [Bureau of Social Welfare and Public Health, Tokyo Metropolitan Government, 2021]; 75°C, HTST) and (3) maintaining the chamber temperature at 63°C for 30 min (for specific heated processed meat products [Ministry of Health and Welfare, Japan, 1948]; used for fish and fishery products [FDA, 2020] to ensure food safety against bacterial pathogens in seafood processing; 63°C, LTLT). Heated samples were incubated at 30°C for 2 h for texture analysis, and stored at the above-mentioned conditions until analysis. This procedure was conducted independently for each fish in this study (n = 3).
Proteins in OM and DM were fractionated according to the method described by Qixing et al. (2014). Briefly, samples were homogenized with 10 volumes of solution A (15.6 mM Na2HPO4, 3.5 mM KH2PO4 [pH 7.5]) and centrifuged at 5,000×g for 15 min. The extraction process was repeated twice. The supernatants were combined and mixed with cold 50% trichloroacetic acid (TCA) to a final TCA concentration of 10%. The resulting precipitate was collected by centrifugation at 5,000×g for 15 min (water-soluble fraction; WSF). The supernatant was used to determine the non-protein nitrogen fraction (NPNF). The pellet was homogenized with 10 volumes of solution B (0.45 M KCl, 15.6 mM Na2HPO4, 3.5 mM KH2PO4 [pH 7.5]) and centrifuged at 5,000×g for 15 min. The extraction process was repeated twice. The supernatants were combined to form the salt-soluble fraction (SSF). The precipitate was stirred with 10 volumes of 0.1 M NaOH for 2 h. The mixture was centrifuged at 5,000×g for 15 min with the resulting supernatant becoming the alkali-soluble fraction (ASF), and the final residue was used to determine the alkali-insoluble fraction (AIF). The nitrogen content in each fraction was determined by the Dumas combustion method using a Vario Max CN nitrogen analyser (Elementar Analysensysteme GmbH, Hanau, Germany). The data are presented as the protein composition ratio (%), which is the ratio of nitrogen content of each fraction to the sum of nitrogen content of all fractions.
SDS-PAGE was performed on 10% gels (e-Pagel, Atto, Tokyo, Japan) according to the method described by Laemmli (1970). A total protein fraction (TF) was extracted according to a method described by Numakura et al. (1985) with slight modifications. After chopping, filet tissue samples were heated at 100°C for 10 min in solution C (8M urea, 2% SDS, 2% 2-mercaptoethanol, 20 mM Tris-HCl buffer [pH 8.8]) and stirred for 20 h. After centrifugation at 12,100×g for 30 min, supernatant samples containing 5.0 μg protein were separated by electrophoresis. WSF and SSF samples were also separated by SDS-PAGE. SSF samples were mixed with cold 50% TCA to a final TCA concentration of 10%, and centrifuged at 10,000×g for 5 min. In addition to WSF, these pellets were washed with acetone to remove residual TCA and re-dissolved in solution D (8M urea, 4% SDS, 5% 2-mercaptoethanol, 25 mM Tris-HCl buffer [pH 6.8]). The supernatants were mixed with the same volume of SDS-PAGE sample buffer (4% SDS, 10% 2-mercaptoethanol, 20% glycerol, 0.005% bromophenol blue in 0.125 M Tris-HCl buffer [pH 6.8]) and heated at 95°C for 5 min. The samples, each containing 5.0 μg protein, were loaded into each lane. After electrophoresis, the gels were stained with Coomassie Brilliant Blue.
DSC analysis was performed using a DSC-60Plus thermal analyser (Shimadzu, Kyoto, Japan) with distilled water used as a reference. N2 at a flow rate of 30 mL/min was used as carrier gas. Samples (15.0 mg) were weighed and hermetically sealed into aluminium pans using a sealer. The samples were cooled with liquid nitrogen as a cooling medium and scanned from 20°C to 80°C at a rate of 5°C min‒1. The thermal analysis software TA-60WS (Shimadzu) was used to analyse the experimental data. The maximum temperature at each peak was recorded as the maximum peak temperature (Tmax). The denaturation enthalpy (ΔH) was estimated from the area bound by a thermogram and the baseline.
Soluble collagen in the OM and DM was extracted according to the method of Qixing et al. (2014) with slight modifications. In short, samples were homogenized with four volumes of solution E (32.8 mM NaCl, 1.5 mM KCl, 0.5 mM CaCl2), heated for 15 min at 50°C, and centrifuged for 30 min at 2,300×g. Pellets were resuspended in solution E and re-centrifuged. The supernatants were combined (soluble collagen fraction). OM and DM were used to determine the total collagen content (total collagen fraction). After HCl was added to a final concentration of 6 M, the samples were hydrolysed at 130°C for 3 h. The filtered samples were then evaporated. Hydroxyproline content in the hydrolysed samples was analysed according to the method of Woessner (1961), and this number was converted to collagen content using a factor of 9.75 (Zenitani & Yamakita, 1956). Collagen solubility, which is the ratio of soluble collagen amount to total collagen amount, was then calculated.
Results
Fig. 1 shows the changes in protein composition of OM and DM under different heating conditions, based on protein solubility. The NPNF levels of OM and DM were not significantly different among the samples. However, WSF levels and SSF levels in heated samples were significantly lower than those in unheated samples (p < 0.05). The ASF levels in heated samples were significantly higher and lower (p < 0.05) than those of unheated OM and DM, respectively, except for DM samples heated at 63°C. The AIF levels in heated samples were significantly higher (p < 0.05) than those of unheated OM and DM. Among the heating treatments, the ASF and AIF levels in OM after heating at 63°C were higher and lower than those after other heating conditions, respectively, although not significantly. The AIF levels of DM heated at 75 and 85°C were significantly higher (p < 0.05) than those heated at 63°C.
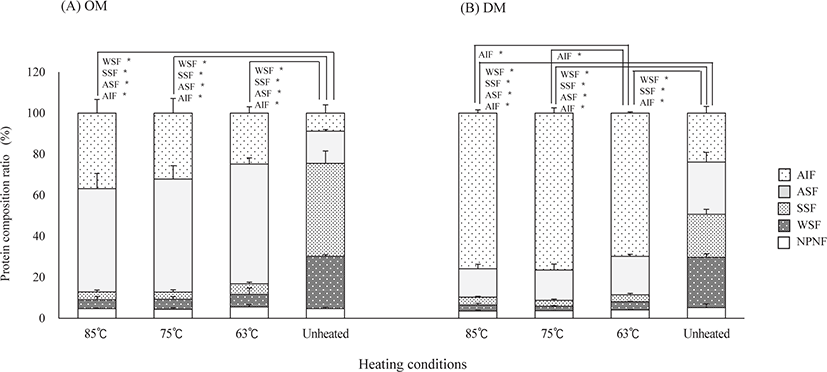
SDS-PAGE banding patterns in OM and DM fractions treated with different heating conditions are shown in Figs. 2 and 3. The TF protein profile was not changed remarkably by heating, indicating that heating under present conditions does not promote protein degradation. In contrast, WSF and SSF banding patterns in OM were noticeably altered by heating. The band intensities around 33 kDa for the WSF, between 180 and 245 kDa, and also at approximately 48 kDa for the SSF, visibly decreased after HTST treatment compared to LTLT. The former (33 kDa) band was consistent with the expected size of tropomyosin (Daifuku et al., 2011), and the latter two bands (between 180 and 245 kDa and approximately 48 kDa) were tentatively identified as myosin and actin, respectively, based on their size (Liang et al., 2012). For the WSF and SSF of DM, the band intensities of proteins were lower in unheated DM than in unheated OM. In addition, the protein bands of both fractions disappeared after heating.
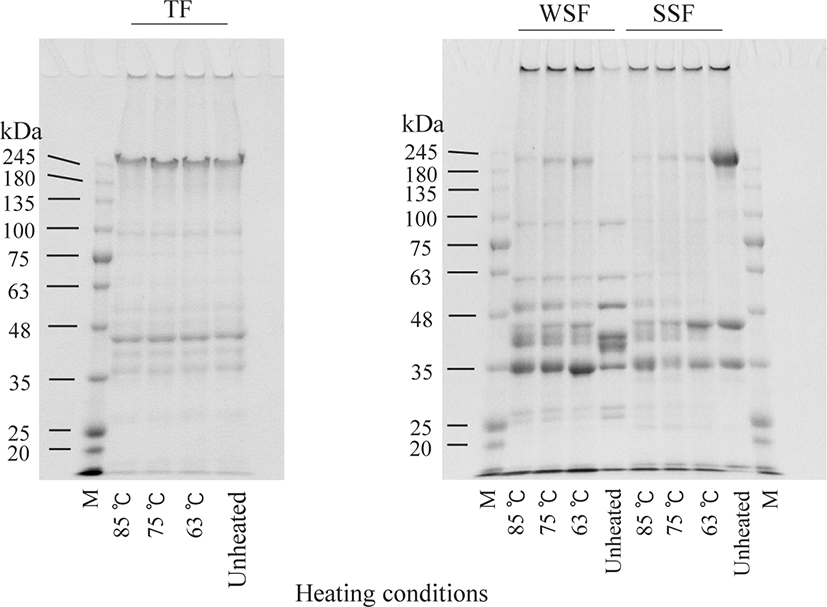
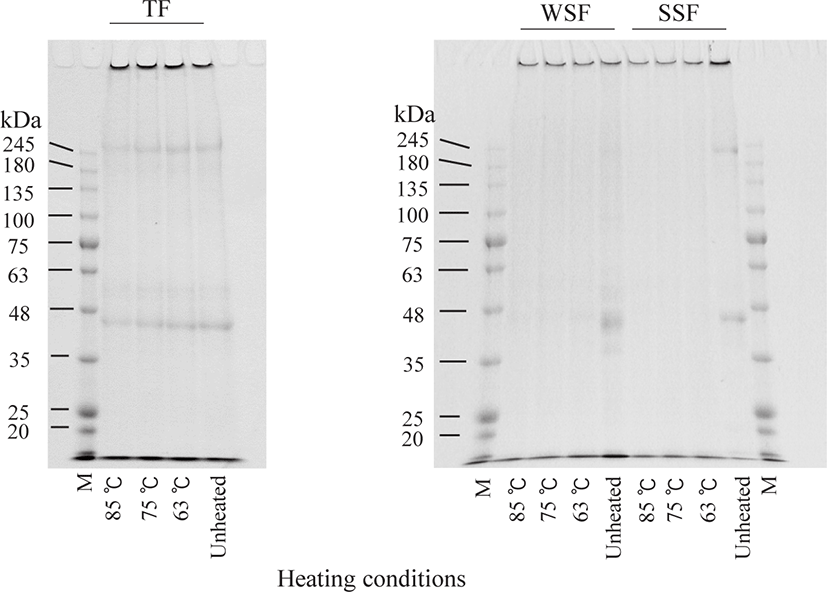
DSC thermograms of OM and DM after heating under different conditions are shown in Fig. 4. Two main peaks, the first peak (peak 1) and third peak (peak 3) observed for the unheated samples mainly corresponded to the denaturation of myosin and other components, and actin, respectively (Akahane et al., 1985). The smaller peak (the second peak; peak 2) was probably related to sarcoplasmic proteins (Hastings et al., 1985). The values of Tmax and ΔH are listed in Table 1. The Tmax of unheated samples was observed at 45.8°C for peak 1, 60.7°C for peak 2, and 71.6°C for peak 3 in OM, and at 49.5°C, 60.1°C, and 71.0°C in DM, respectively. These peaks were not observed in the samples heated at 85°C and 75°C. However, extremely small peaks (peak 3) for OM and DM heated at 63°C were detected at Tmax values of 74.5°C and 72.7°C, respectively.
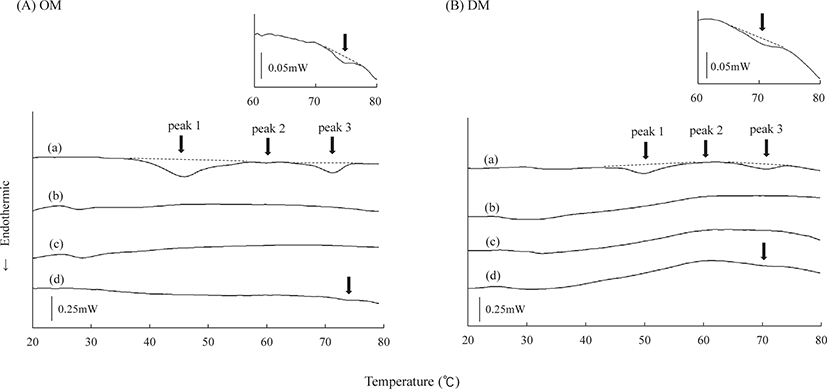
Table 2 shows the collagen content of OM and DM after heating under different conditions. No significant differences in the soluble collagen levels in all OM samples were observed; however, among the heated samples, the collagen from the sample heated at 63°C showed a more increase in solubility compared to the unheated sample. DM showed no significant changes in collagen after heating; however, among the heated samples, the soluble collagen content and collagen solubility of DM heated at 63°C were more increased than those of unheated DM.
Discussion
Among native proteins in fish, sarcoplasmic proteins such as myoglobin are present in the WSF. In contrast, myofibrillar proteins, consisting mainly of myosin and actin, are included in the SSF. Most of these proteins are rapidly denatured during heat treatment, leading to changes in overall protein solubility. Following such heat treatments, these denatured proteins migrate into the ASF or AIF. During heating, the shrinkage of myofibrillar proteins and collagen, which denature and coagulate, cause an increase in the hardness of the meat (Niamnuy et al., 2007). Increasing the heating temperature also accelerates overall protein denaturation in fish. Myofibrillar proteins are specific contributors to fish texture (Ovissipour et al., 2013) and consequently their heating and denaturation results in the meat becoming tougher (Dunajski, 1980).
In this study, the ASF and AIF levels in the OM significantly increased after heating under different conditions, with LTLT showing higher ASF and lower AIF values than those under other heating conditions, although not significantly (Fig. 1). The levels of AIF in all heated DM samples were significantly higher than those in the unheated samples. However, among the heated samples, the levels in DM heated at LTLT were lower than those in other heated samples. Moreover, in contrast to the other samples, the heated samples in DM with LTLT did not show a significant decrease in the levels of ASF after heating. These results suggest that the degree of protein denaturation in DM after LTLT was lower than that of the other heating conditions, and this might have resulted in the more tender texture of the fish after LTLT treatment.
Following this analysis, SDS-PAGE was performed to evaluate the solubility changes of each protein due to denaturation and aggregation during the heating processes (Figs. 2 and 3). The band intensities of some proteins in WSF and SSF in OM heated at higher temperatures were lower than those that underwent LTLT treatment. This suggests that treatments at higher temperatures resulted in increased denaturation of these proteins more (Qixing et al., 2014) and simultaneously altered their solubility more. In particular, for the SSF in OM, the band at approximately 48 kDa, identified as actin, was more intense after LTLT than after HTST. These results indicate that the solubility change of actin in OM due to protein denaturation did not occur after heating with LTLT conditions when compared with that under other heating conditions. The band around 33 kDa for the WSF in OM was consistent with tropomyosin which is a highly heat-stable protein (Woods, 1969). In fish muscle, actin is bound to thin filament proteins, such as troponin and tropomyosin (Godiksen et al., 2009). The band around 33 kDa, as well as the band around 48 kDa, decreased in intensity after HTST compared to LTLT (Fig. 2). Therefore, it appears that the solubility changes in tropomyosin, as well as actin, were a result of the different heating conditions. However, Samejima et al. (1982) concluded that tropomyosin does not influence the gel texture of meat. Thus, the structural change of tropomyosin due to heating of fish may not be related to the textural changes that we observed. On the other hand, the protein bands observed in the WSF and SSF of unheated DM samples disappeared after heating (Fig. 3); therefore, it was difficult to compare the protein compositions of DM after heating under different conditions.
During DSC analysis, slight peaks were observed in the OM and DM samples at 63°C, suggesting that actin was not partly denatured after heating (Fig. 4 and Table 1). Ishiwatari et al. (2013a) reported that the effect of myosin denaturation of meat on the elastic modulus is small, while that of actin denaturation has a remarkable impact. Namely, heating at a higher temperature than that required for thermal denaturation of actin causes the muscle fibres to contract, resulting in firmer meat (Tornberg, 2005). This means that the denaturation level of actin affects the whole structure of myofibrillar proteins; in other words, incomplete denaturation of actin leads to more tender heated meat (Ishiwatari et al., 2013b). Thus, the difference in the degree of actin denaturation may be one of the reasons why the meat heated with LTLT in this study was generally more tender and may also explain why muscle contraction of fish was suppressed in our previous study (Furuta et al., 2020).
In addition, collagen solubilization of OM and DM showed a tendency to increase with LTLT treatment (Table 2). Collagen fibres treated at temperatures above 60°C lose their native structure and begin to solubilize (Dunajski, 1980; Kanoh et al., 1988). Additional heating causes the collagen to dissolve and gelatinize (Tornberg, 2005), resulting in more tender cooked fish (Hatae et al., 1996). Moreover, collagen solubility influences the texture of cooked meat products (Eilert & Mandigo, 1993). On the other hand, Kanoh et al. (1988) suggests that collagen is solubilized during heat treatment; however, this phenomenon might have been masked by the denaturation of myofibrillar proteins. Therefore, in this study, collagen solubilization might have contributed to a more tender texture of cooked fish after LTLT, but may not be the main cause of textural change.
Fish muscles are mainly composed of OM. Thus, the texture and flavour, i.e., palatability, can be attributed primarily to OM. However, it is unclear how the composition ratio of DM in fish muscle affects the quality of heated fish, and further studies are needed to elucidate this. The results of the present study support that LTLT heating is an excellent condition for the maintenance of the tenderness of cooked fish (Furuta et al., 2020). Therefore, high-quality fish products subjected to LTLT treatment are expected to contribute to the increase in the consumption of fish.
In conclusion, the results of protein solubility, SDS-PAGE, and DSC analysis suggest that, during heating conditions set to prevent food poisoning, the degree of actin denaturation in fish with LTLT was smaller than that under other heating conditions. In addition, the solubility of collagen tended to be higher after LTLT than after the other treatments. These results indicate that the different degrees of denaturation of actin and collagen may be responsible for increased tenderness texture and lower fish shrinkage with LTLT compared to treatments at higher temperatures. However, macroscopic structural changes such as contraction of myofibrils and changes in collagen fibres have not yet been elucidated and need to be studied to confirm the factors that lead to differences in the texture of fish under different heating conditions.